When I moved from Brazil to the United States to train as a neuroscientist, I was shocked to discover that most of our ‘knowledge’ about the human brain in fact came from another species: the mouse. This struck me as pretty strange. After all, it wasn’t the mouse brain that put us on the Moon or that decoded the human genome. It was the human brain in all its complexity that generated our understanding of life and of the laws that govern the Universe.
Nonetheless, as a postdoctoral researcher, I set about busily learning how to dissect mouse brains. I hoped to find out more about the major regions and important structures associated with mental and neurological disorders such as autism and epilepsy – and, ultimately, how to fix them. I practised and practised, until I was finally an expert in the anatomy of the mouse nervous system. But it was bloody work, and I paid the price at night. In my dreams, I replayed the vivid experience of removing the brains from tiny skulls and slicing them up. Something about these nightmares was telling me not to continue down this road. Eventually, I mustered the courage to challenge my colleagues: what if the diseases we want to cure, and the answers we want, won’t be found in the mouse brain?
Many scientists would remind me of how similar these two species are in terms of brain structure. However, despite their similarities, these two species took distinct evolutionary paths, adapting the brains for their respective lifestyles and evolutionary niches. This explains why cures in mice don’t always translate into cures for humans. But there’s a reason why neuroscientists tend to rely on mouse models – it’s more practical than testing on people. Historically, however, it’s left us with many unanswered questions, such as when does the first human neuron fire? And when does the human cortex form?
We do, at least, know when the human cortex – the brain region that’s related to our most sophisticated types of cognition – forms: it grows in utero. While we normally use non-invasive techniques such as ultrasound to visualise the developing foetal brain, we can’t experimentally investigate it at a resolution that allows us to understand it. The limitations aren’t just technological – there are obvious ethical concerns about invasively studying a human embryo inside the womb.
But what if we could recreate the human brain outside the uterus? In 2008, the Japanese researcher Yoshiki Sasai showed that it was possible to cultivate artificial human neural tissues outside the womb. So-called ‘pluripotent’ stem cells have the ability to generate cell types for all tissues in the human body. You can find pluripotent stem cells in the human embryo, or by reprogramming cells from a more developed organism, which captures the genome of the donor. Independent of its origins, Sasai has shown that one can guide these cells to become a neural tissue by exposing them to specific external factors. When suspended in liquid, these neural-committed cells will spontaneously self-aggregate, and form small, organised three-dimensional clusters that resemble the anatomic structure of the foetal human brain. We now call these structures ‘brain organoids’ – ‘mini-brains’ in pop culture.
These brain organoids can grow up to 0.5 cm, a pea-sized piece of white tissue that you can actually visualise with the naked eye. We keep them floating in a juicy, reddish stew that contains all the nutrients the cells need to survive. And, indeed, they can survive for weeks, months and years. Our record to date is three full years – and it seems we could keep them even longer than that, as long as someone keeps replacing the juice. The beauty of these organoids is that they re-enact the way that the human brain develops in the womb. Contrary to a mouse brain that’s fully formed in around 20 days, it takes nine months for a human brain organoid to become similar at the molecular, cellular and functional level to that of a newborn baby brain.
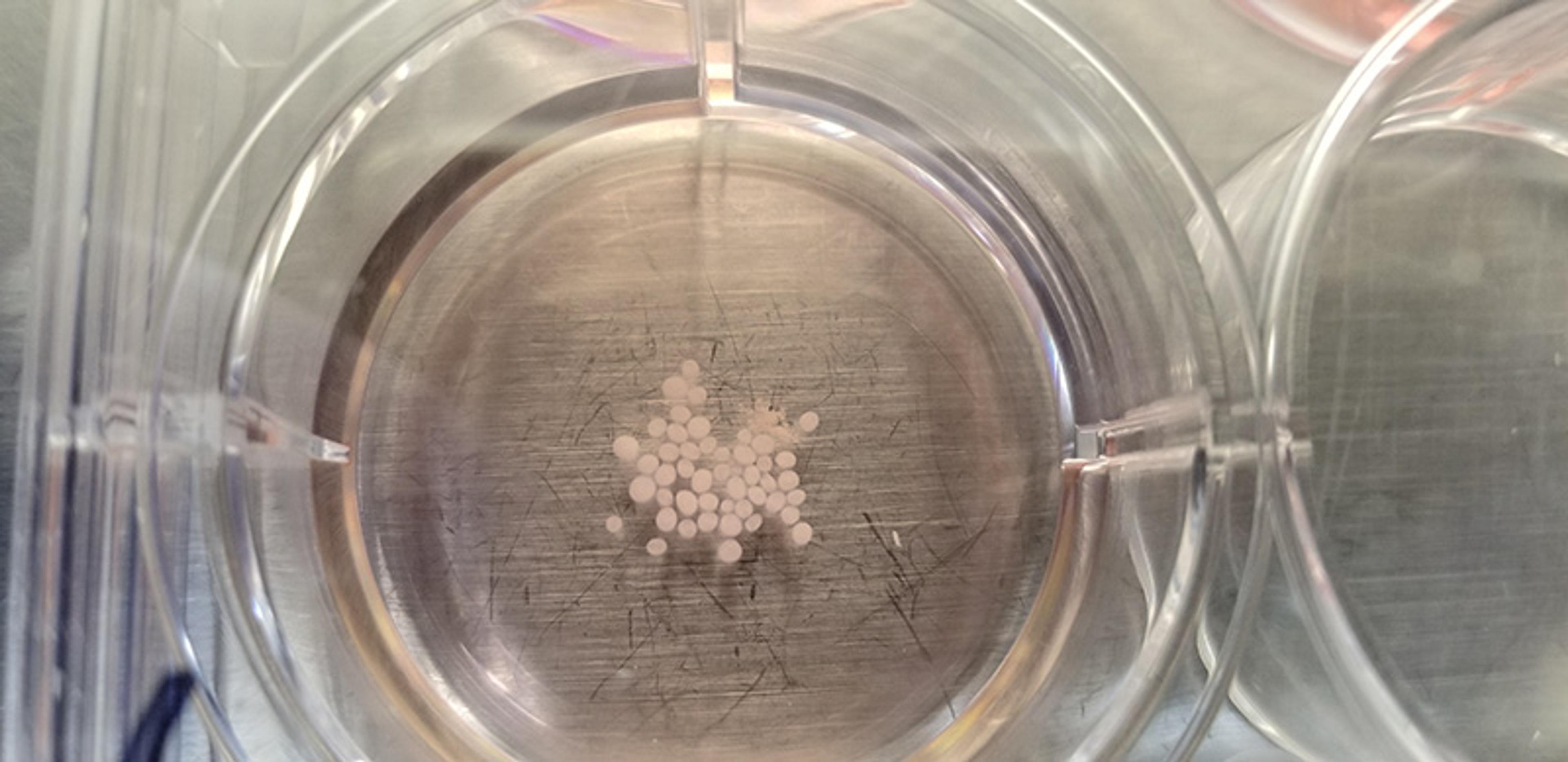
Brain organoids created in a petri dish. Each white sphere is a human brain organoid, composed of around 2.5 million neurons, derived from pluripotent stem cells. Brain organoids can last for several years and grow up to 0.5 cm in diameter
But don’t get too excited just yet. These aren’t actually miniaturised human brains in a dish. These structures lack certain cell types, there’s no blood supply, and they lack input from other parts of the brain and from other non-brain tissues in the body. Moreover, they’re tiny, with no more than 2.5 million neurons (compared with 86 billion neurons in the human brain). Nonetheless, even with these limitations, this reductionist human brain model was crucial in helping my team figure out that the Zika virus was the culprit behind the outbreak of birth defects in the northeast of Brazil in 2015. My lab has also used this tool to find novel treatments for some rare neurological disorders with no cure. Not bad for a tiny brain.
As scientists have built on Sasai’s initial discovery, we now have organoids that are even better at mimicking the molecular and cellular components of the human brain. However, as important as this technology seems, there was still something missing: brain organoids still wouldn’t display the same neural network activity as the human brain.
During development, millions of neurons are being produced every day and, as they mature, they start forming connections to each other. These connections aren’t formed randomly; rather, they will eventually form what’s known as a structured neural network. The orchestration of these networks was thought to involve signalling from different brain regions and even non-neural tissues. Thus, it seemed unlikely that we’d observe this type of neural network in human-made brain organoids in a dish. Researchers would blame their intrinsic limitations: based on what we know from studying the mouse brain, these structured networks should appear only in a fully formed brain inside an organism. It wouldn’t be possible to recreate sophisticated brain activity in a dish.
Trying to understand autism by studying a brain without neural activity is like trying to understand diabetes by studying a pancreas without insulin
This posed a problem for me both personally and professionally. The conditions that my lab currently examines on are caused by perturbations in structured neural networks, such as schizophrenia and autism. I have a deep, lived understanding of these illnesses that comes from the fact that my son was born with profound autism. He is non-verbal, with severe developmental delay and hundreds of seizures per week. He frequently engages in stereotyped and addictive behaviour – he is able to spend hours manipulating a single object, rocking in a chair or persistently trying to accomplish an improbable task. Still, his mind is extraordinary when it comes to exploring the surrounding environment. He can spot a new item in a big store as if it was screaming his name. A Christmas light decoration can seem as fascinating as an exploding nebula. Against this backdrop, the idea of trying to understand autism by studying a brain without neural activity would be like trying to understand diabetes by studying a pancreas without insulin.
This limitation is what first encouraged us to find ways of allowing brain organoids to mature over time. After keeping brain organoids alive for several months, we finally observed the spontaneous emergence of brain oscillatory waves, similar to those detected by electroencephalograms (EEG). These EEG-like oscillatory waves are ubiquitous in all living human brains, but were never before recorded emerging from any living human-made system. It was the final proof that this simplistic model of the human brain could, indeed, in some ways recreate the organised formation of the networks that arise in the womb. Because autism is believed to start in utero, we’re now applying this technology to understand how perturbations of these neural oscillations might cause autism. The research will hopefully lead us to better interventions for my son, and millions of other people living with autism spectrum disorders worldwide.
The appearance of EEG-like waves in human brain organoids was, no doubt, a major milestone, but it also triggered ethical and moral concerns. The new technology that lets organoids develop in this way also allows them to incorporate input and output from other brain regions or sensory information, such as human stem cell-derived retina or pain sensors. What if these organoids acquire some level of consciousness or self-awareness? While the goal of purposely creating consciousness using human brain organoids isn’t the focus of most investigators, consciousness might unintentionally emerge as techniques for disease modelling improve.
Ethical quandaries can arise long before any human-level consciousness is achieved. The extent to which organoids are conscious and possess other cognitive capacities will be vital in determining their moral standing. While organoids don’t yet exhibit anything like human-level consciousness, we can’t rule out the idea that they will at some point display evidence of it as observed in a human brain. We’ve set up a couple of experiments to determine if any human-level consciousness is present in more sophisticated brain organoids. For example, treatment with certain anaesthetics might eliminate the oscillatory waves in these organoids, establishing a correlation with a lack of consciousness in human subjects. This will, undoubtably, put a pause on research. It would be necessary to grant these organoids some sort of moral status, and we’ll need to learn how to handle this issue as we go forward.
Putting ethical concerns to one side, complex networks in human brain organoids might reveal one of the greatest mysteries of the human brain: how do we learn? Learning is a complex cognitive process, shaped by evolution over millions of years and modulated by the nervous system in real time. An essential step towards understanding human cognition involves dissecting the fundamental biological mechanisms of learning. As the brain matures, it shifts from an experience-deprived embryonic brain, to a constantly stimulated sensorial brain after birth. Previous investigations of the biological mechanisms of learning focused only on postnatal subjects, and ignored the critical neurodevelopmental period when this transition happens in utero.
The short-term memory of chimpanzees is orders of magnitude more powerful than of adult humans
We’ve recently extended the brain organoid model to capture this critical period of early learning. To do so, we designed a four-legged robot that walks using the coordinated electrical activity from a brain organoid – a brain on legs. The robot has infrared sensors, detecting obstacles before hitting them. When it approaches an object, the robot stimulates the organoids. In response, they activate a new set of neurons that, when detected by a computer, can give a ‘turn right’ command to the robot. This provides sensorimotor feedback to the organoid as it explores the environment, to evaluate if the organoid can learn from a set of pre-determined variables, such as a simple obstacle course.
We anticipate that this robotic system will adapt, evolve and create solutions based on what obstacles it encounters. Through these experiments, we hope to shed light on the mechanisms of learning in early neurodevelopment, and to set the stage for future investigations in systems neuroscience and embodied cognition. Dissecting how an organism adjusts its sensorimotor and nervous systems is not only crucial for understanding human intelligence and creativity, but also for designing more robust and efficient learning algorithms for AI research.
Such research isn’t limited to developing a more human-like AI, but could be applied to the neural networks resembling any living organism. Our lab has an entire brain organoid ‘zoo’, created to capture neural networks that outsmart the human brain. For example, the short-term memory of a chimpanzee is several orders of magnitude more powerful than the short-term memory of adult humans. While the reasons for that pre-eminence are currently unclear, we can make the most of these diverse evolutionary pathways and abilities to create an AI that’s more suitable for specific applications, even for those beyond this planet.
In the summer of 2019, my group sent the first batch of human brain organoids to the International Space Station (ISS). The goal of this and other upcoming space missions is to measure the influence of outer space on the human brain. What we learnt from studying astronauts who lived on the ISS for long periods of time is that microgravity and space radiation can have a negative impact on the physiology of the human body. We didn’t evolve to be up in space, and perhaps one of the most important consequences of exposure is the effect on cognition. Understanding how human brain cells react to this novel environment will help us design new strategies to mitigate the negative effects of long-term space travel and perhaps even enable interplanetary colonisation in the future. The research will also teach us about human ageing, and help us develop better treatments for neurological conditions such as Alzheimer’s disease and dementia.
Finally, scientists are also using brain organoids to understand key evolutionary questions about the origins of the human brain. I have an abiding fascination for the Neanderthals that’s been with me since I was a child, and raises deep philosophical questions that continue to haunt me: who are we? What makes us human? Neanderthals weren’t as sophisticated in terms of arts, technology and adaptation as modern humans, even though their brain’s volume was similar to ours. Neanderthals shared part of their evolutionary story with us, we mated, and then they disappeared. Understanding how these two populations interacted would tell us a lot about ourselves.
Just over 150 years ago, the first fossils of the Neanderthals were discovered in Germany. By analysing these bones and comparing them with ours, we can get an accurate picture of what the Neanderthals might have looked like, when their lineage split from our own, and when they went extinct – about 40,000 years ago. In 2010, the first draft of the Neanderthal genome was published based on ancient DNA samples collected from the bones of three female individuals, who lived at different times in a cave in Croatia, about 40,000 years ago. This was followed by a more complete genome sequence of an archaic hominin from Denisova cave in the Altai mountains of Siberia. Genetic analysis of the Denisovan individual revealed that its population is a sibling group to Neanderthals.
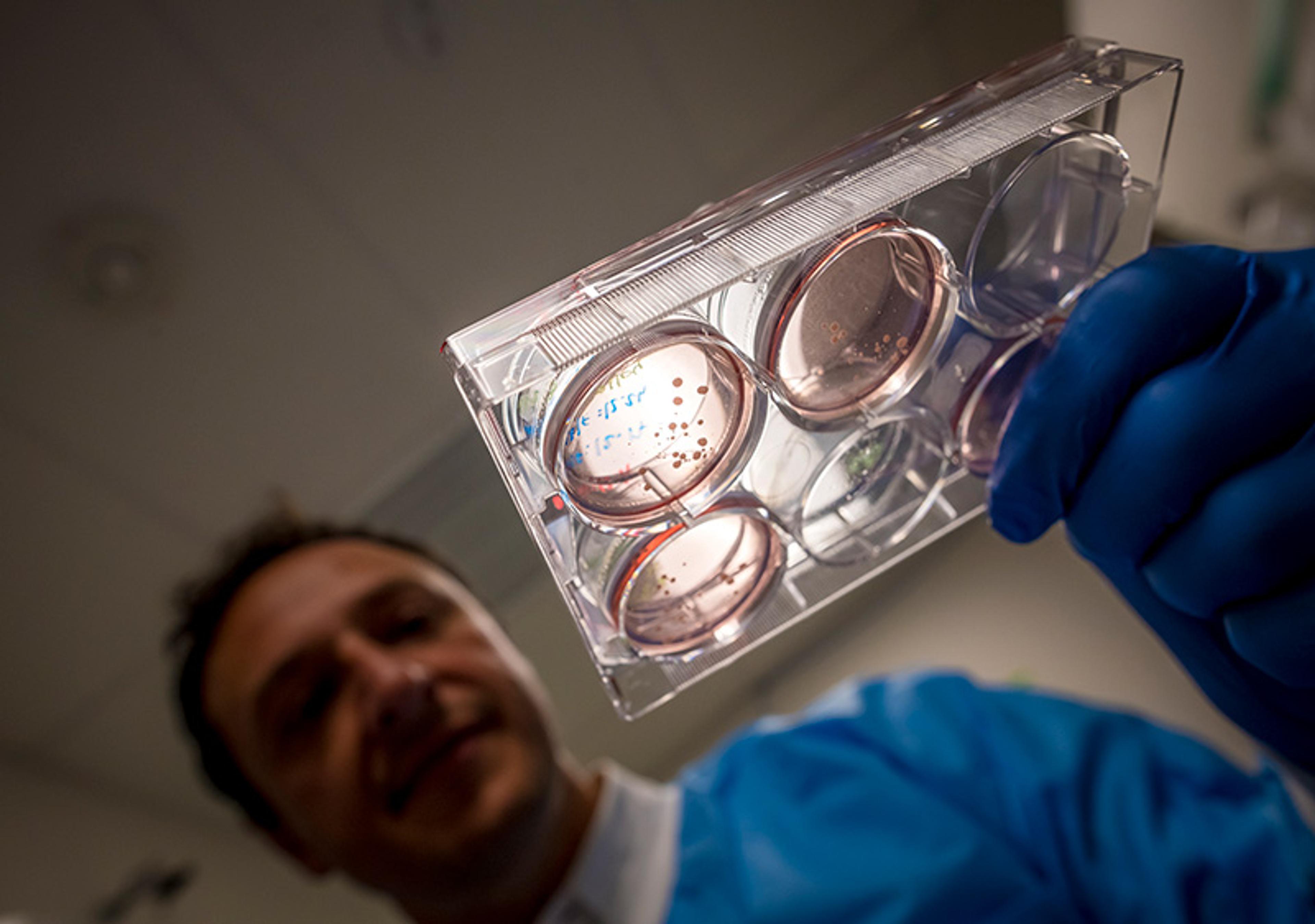
Alysson Muotri holding a plate with ‘archaised’ human brain organoids, carrying an extinct genetic variant that is no longer present in modern human genomes, but was present in Neanderthals and Denisovans, our closest extinct relatives
Comparing Neanderthal, Denisovan and extant human genomes has revealed that many modern humans carry genes introduced from interbreeding. It’s also allowed us to identify human-specific genetic differences that might have been important for our more recent evolution. Human genomes have much in common with Neanderthal and Denisovan genomes. To date, the primary focus of comparative genetics between archaic and modern humans has been on these shared, or introgressed, sites, where modern humans have adopted archaic elements. In this way, groups of Homo sapiens arriving in a new area were able to co-opt some of the adaptations that other groups of humans had developed over millennia of natural selection. Examples range from immunity to local pathogens to physiological adaptations for living at a high altitude.
Understanding the evolutionary path to modern humans could illuminate the origins of human disease
Comparative genetics has also shown that we’ve inherited susceptibility to certain conditions or diseases from our extinct relatives. Diabetes is one of them. While insulin resistance might be beneficial in a starving environment, it creates all kinds of problems for the body when food is plentiful and accessible. More intriguing is the finding that addiction is also something that we acquired from our extinct relatives. Addiction could be a survival factor when the situation requires repetitive behaviour to accomplish something with a low probability – but when drugs that act on our reward system are easily available, we suffer its consequences.
While a great deal of interest has been placed on the clinical benefits and consequences of understanding the genes we share with our ancestors, my team and I argue that areas of divergence could be of equal or greater clinical value. Unfortunately, this runs up against the common misconception that evolutionary studies won’t translate into medical benefits. For example, while the mechanisms behind humans’ unusual bipedal posture and striding gait remain hotly debated, it’s clear that several diseases – such as hernias, haemorrhoids, varicose veins, spine disorders, knee joint osteoarthritis, uterine prolapse and difficult childbirth – all arose from this marked change in anatomy and organ physiology. Understanding the evolutionary path to the modern human is likely to illuminate the origins of human disease.
To find the regions in the genome that are uniquely ‘us’, we compared the genomes of our extinct relatives with those of modern humans. Surprisingly, by looking at protein-coding genes, we found only 61 genes that are different between the Neanderthals and us. From those 61, a handful are directly related to the brain, and only one seems to be relevant at the very early stages in human neurodevelopment. This is a gene called NOVA1. It’s important, because it regulates the activity of hundreds of other genes downstream, mostly the ones implicated in synapses, the structures that allow for communication between brain cells. The difference is in a single DNA letter, or base pair, enough to distort a bit of the protein and change the way it functions inside the brain cells. But how to test this?
Our approach was to use genome-editing enzymes to convert the NOVA1 modern gene into an archaic version – to ‘neanderthalise’ the modern human genome in pluripotent stem cells and generate brain organoids from them. We now call these brain organoids ‘neanderthoids’. Interestingly, we can visualise the differences in the shape of the organoids carrying the archaic and modern versions. Inspection at molecular level confirmed our hypothesis: several NOVA1 target genes were affected, altering the composition of the synapses. At the functional level, we observed that the networks on brain organoids carrying the archaic NOVA1 version showed a higher activity at earlier stages. The changes of neural networks in these ‘neanderthalised’ brain organoids parallel the rapidly acquired abilities observed in primate neonates when compared with human newborns. A baby chimp can outsmart a human newborn but this changes as we develop. This is highly speculative, but our data might suggest that the DNA alteration in NOVA1 that we all carry now in our genomes could be a turning point in human evolution, providing a more complex and sophisticated brain at the cost of postnatal human care – a very provocative and intriguing idea.
Of course, this type of research has limitations. One of them is related to the level of alteration required to draw more robust conclusions. For example, what about the other 60 genes? How would the brain organoids develop with all these alterations? To explore this, we created the Center for Archealization (ArchC) at the University of California San Diego, where a multidisciplinary team will move forward with this proof-of-concept. The final goal is to catalogue the relevant genetic variants that make us unique. This transformative strategy will illuminate not only how DNA alterations in key genes lead to a highly sophisticated brain, but also shed light on the origins and causes of mental illness.
We have now tools to answer many mysteries of the human brain and perhaps some of the deepest philosophical questions that confront humanity. The tools aren’t perfect, and there’s plenty of room for improvement. Still, even with the approaches that we now have to hand, it’s possible to better understand how our brains work and to apply that knowledge to several other applications. As long as we keep our eyes and minds open, these tiny human brains are ready to teach us a lot about ourselves, and about our future as a species.